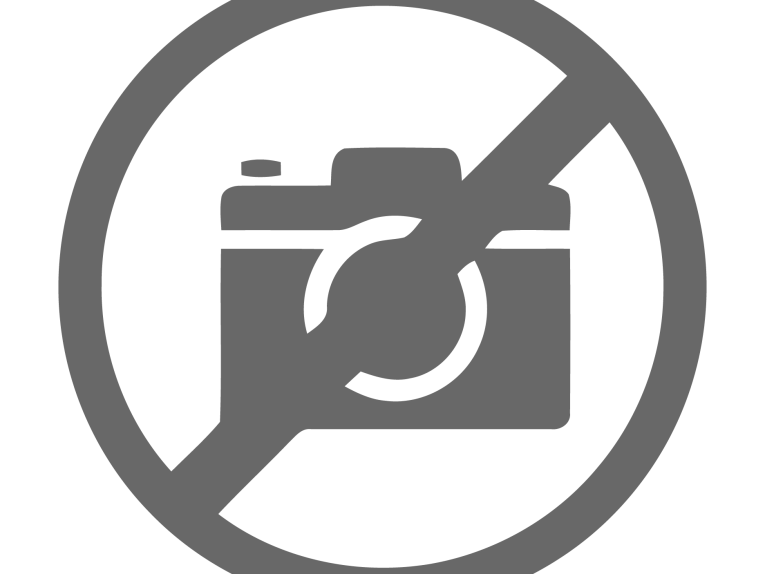
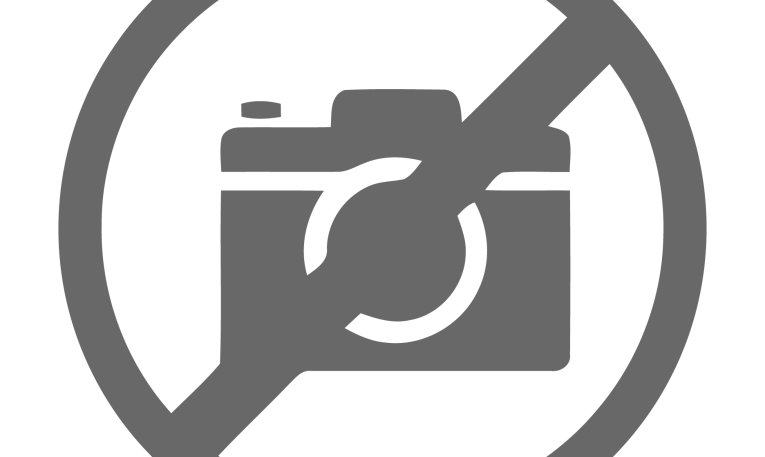
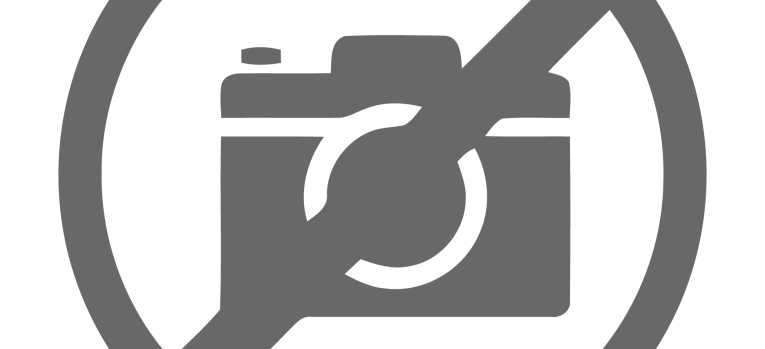
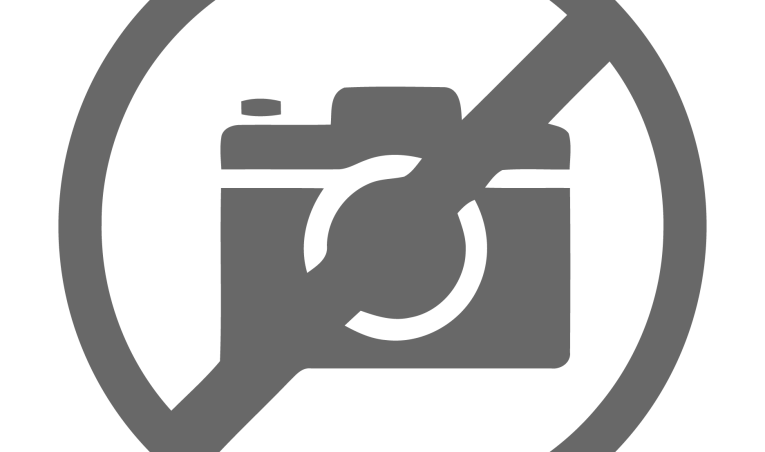
Applications for the PD.124NR1 include use as a midbass driver in multiway systems or as a dedicated woofer bass reflex or horn-loaded designs. The feature set for the PD.124NR1 is like most high-performance pro sound drivers, fairly substantial. Starting with the frame, the PD.124NR1 uses a proprietary six-spoke cast-aluminum frame incorporating six 30 mm × 2 mm rectangular vent holes in the area below the spider mounting shelf for enhanced voice coil cooling. This series of cooling vents allows air to move past the voice coil and across the front side of the neodymium motor assembly.
The cone assembly consists of a curvilinear paper cone along with a 5” diameter paper dust cap. Compliance is supplied by pleated coated cloth M-type surround and from two silicone 6” diameter flat spiders (dampers) mounted back-to-back on an aluminum standoff at the base of the frame. The PD.124NR1’s motor design is unique, and utilizes a radial charged neodymium magnet structure. The neodymium magnet motor was FEA-designed using a 101.6 mm (4”) diameter voice coil wound with round copper clad aluminum wire (CCAW) on a non-conducting glass fiber former. Motor parts, such as the return cup and rear heatsink plate are coated with a black heat emissive coating for improved cooling, with the cooling additionally enhanced by a 35 mm flared pole vent (flare extends to 60 mm), plus six 10 mm diameter vents over the vertical placed neodymium magnets. Self-inductance is controlled by an aluminum demodulation ring (shorting ring or Faraday Shield). Last, the voice coil is terminated to terminal block molded into the frame and features a color-coded pair of push terminals.
I commenced testing of the PD.124NR1 using the LinearX LMS analyzer and VIBox to create both voltage and admittance (current) curves with the driver clamped to a rigid test fixture in free-air at 0.3V, 1V, 3V, 6V, 10V, 15V, 20V, and 30V, allowing the voice coil to progressively heat up between sweeps with a 200 Hz sine wave. The PD.124NR1 remained sufficiently linear enough for LEAP 5 to curve fit at the 30 V level, so all the data was utilized. Following my established protocol for Test Bench testing, I no longer use a single added mass measurement and instead use the measured Mmd data (52.8 grams for the PD.124NR1). I post-processed the 16 550-point stepped sine wave sweeps for each PD.124NR1 sample and divided the voltage curves by the current curves to generate impedance curves, with the phase derived using the LMS calculation method. I then imported them, along with the accompanying voltage curves, to the LEAP 5 Enclosure Shop software.
Because Thiele-Small (T-S) parameters provided by the majority of OEM manufacturers are generated using either the standard model or the LEAP 4 TSL model, I additionally created a LEAP 4 TSL parameter set using the 1 V free-air curves. I selected the complete data set, the multiple voltage impedance curves for the LTD model and the single 1 V impedance curve for the TSL model in the Transducer Model Derivation menu in LEAP 5 and created the parameters for the computer box simulations. Figure 1 shows the 1 V free-air impedance curve. Table 1 compares the LEAP 5 LTD and TSL data and factory parameters for both of Precision Devices PD.124NR1 samples.
At first glance, LEAP 5 parameter calculation results for the PD.124NR1 appear to be very different, however, that is not the case. The fs/Qt ratios are very close, such that when I programmed the factory TSP in LEAP with the same box simulation, the factory curve exactly overlaid the curves done with my measurements.
The only real differences were Precision Devices uses a slightly more conservative Sd calculation, plus Precision Devices also uses 1 W/1 m for sensitivity instead of my 2.83 V/1 m, which accounts for the higher number on their datasheet. Also the published coil length and gap height dictate a 5.5 mm Xmax, so the quoted 8.5 mm is, like a lot of other pro sound manufacturers (e.g., B&C Speakers), specifically accounting for the gap area fringe field, which I certainly understand and have no problem with.
Following my established measurement protocol, I configured computer enclosure simulations using the LEAP LTD parameters for Sample 1. Two computer box simulations were programmed into LEAP 5—one the factory recommended vented box with a 1.6 ft3 volume (15% fill material) tuned to 60 Hz and a Chebychev/Butterworth vented alignment with a 1.3 ft3 volume tuned to 68 Hz, also simulated with 15% fiberglass damping material.
Figure 2 displays the results for the PD.124NR1 in the two vented enclosures at 2.83 V and at a voltage level sufficiently high enough to increase cone excursion to Xmax + 15% (6.1 mm for the PD.124NR1). This produced a F3 frequency of 69 Hz (F6 = 53 Hz) for the factory recommended enclosure and -3 dB = 73.4 Hz (F6 = 59 Hz) for the Chebychev/Butterworth vented simulation. Increasing the voltage input to the simulations until the maximum linear cone excursion was reached resulted in 126 dB at 90 V for the factory recommended box and 127 dB for the same 90 V input level for the smaller vented box.
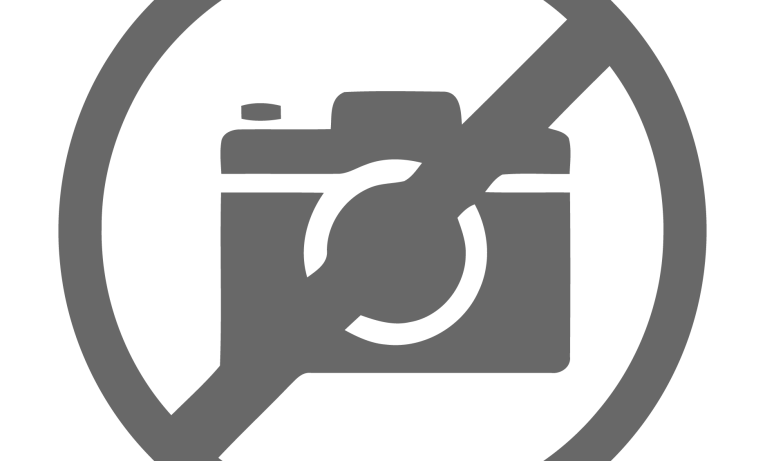
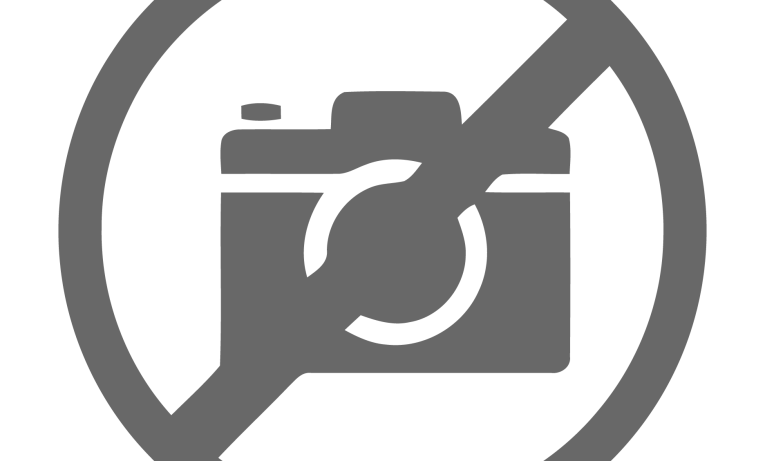
Klippel analysis for the Precision Devices 12” pro sound woofer produced the Bl(X), Kms(X) and Bl and Kms symmetry range plots given in Figures 5-8. (Our analyzer is provided courtesy of Klippel GmbH, and analysis is performed by Pat Turnmire, owner of Redrock Acoustics and the author of the SpeaD and RevSpeaD transducer development software.)
The Bl(X) curve (see Figure 5) is rather broad and symmetrical with some small amount of offset. Looking at the Bl symmetry plot (see Figure 6), this curve shows a trivial 0.5 mm coil-in offset at the 5.5 mm physical Xmax position. The offset set remains constant throughout the operating range of this driver.
Figure 7 and Figure 8 show the Kms(X) and Kms symmetry range curves for the PD.124NR1. The Kms(X) curve is also rather symmetrical in both directions accompanied by a small amount of coil-out offset. Looking at the Kms symmetry range plot, the coil-out offset at the driver’s 5.5 mm physical Xmax is only 1.2 mm. Displacement limiting numbers, calculated by the Klippel analyzer for the PD.124NR1, were XBl @ 82% Bl = 7.2 mm and for XC at 75% Cms was 5.3 mm (nearly at Xmax), which means that for this driver, the compliance is the most limiting factor for prescribed distortion level of 10%, so in a two-way application, quite good. If you apply the less conservative 20% criteria, XBl goes to 9 mm and XC changes to 9.5 mm, so even better.
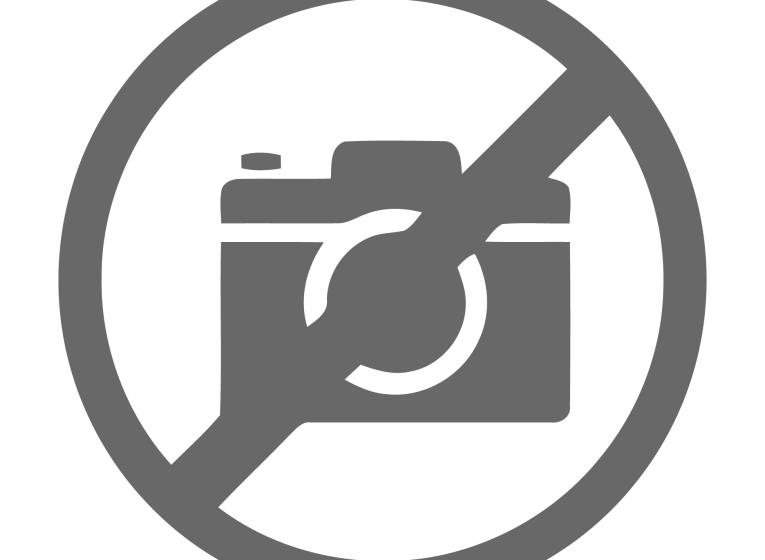
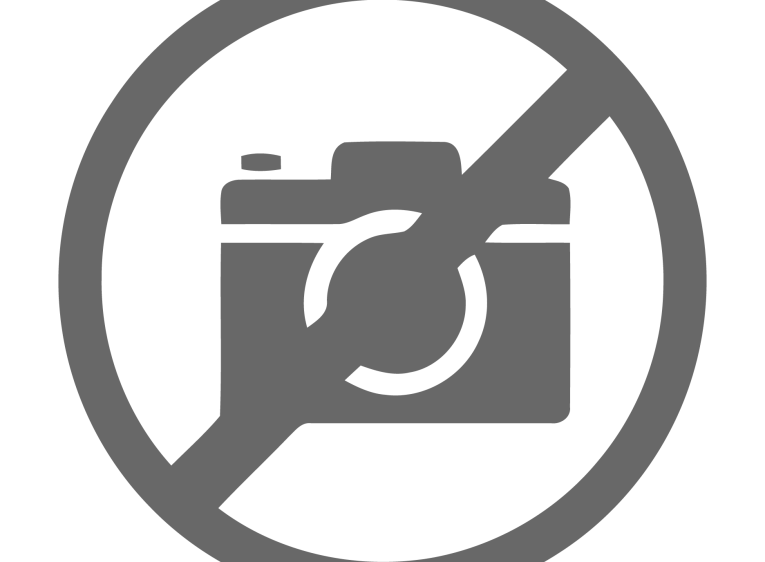
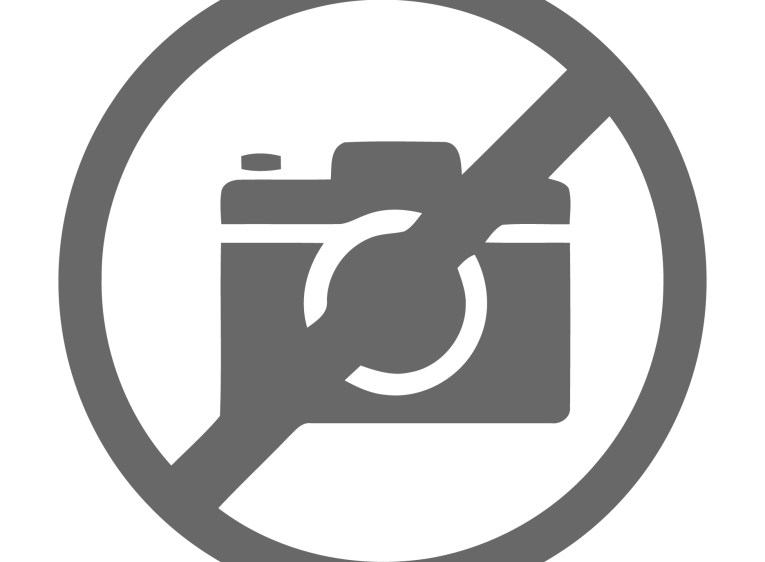
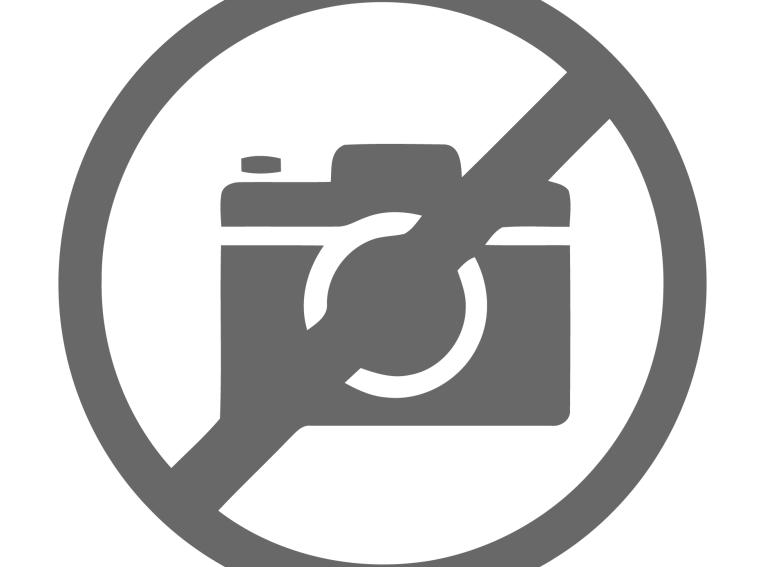
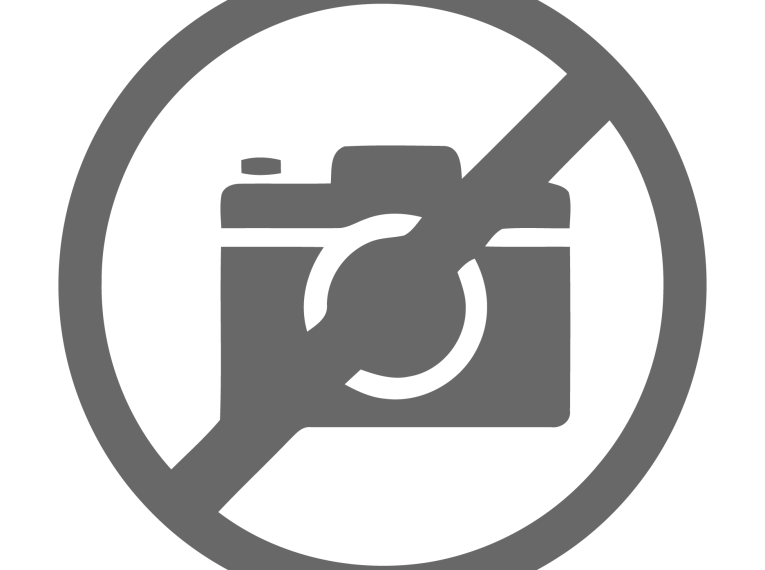
Figure 9 gives the inductance curves Le(X) for the PD.124NR1. Inductance will typically increase in the rear direction from the zero rest position as the voice coil covers more pole area, which is not what is happening here, but is typical of this type of neodymium motor (radial magnet placement). The inductance swing for this driver, which has an aluminum shorting ring, is a really low 0.1 to 0.02 mH, which is excellent performance.
Next, I mounted the PD.124NR1 woofer in a foam-filled enclosure that had a 15” × 14” baffle and then measured the device under test (DUT) using the Loudsoft FINE R+D analyzer (courtesy of Loudsoft) and the GRAS 46BE microphone (courtesy of GRAS Sound & Vibration) both on and off-axis from 200 Hz to 20 kHz at 2 V/0.5 m normalized to 2.83 V/1 m, using the cosine windowed Fast Fourier Transform (FFT) method.
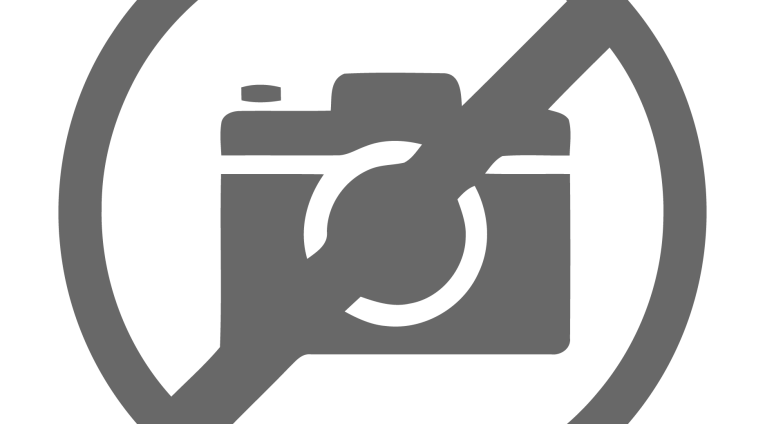
response.
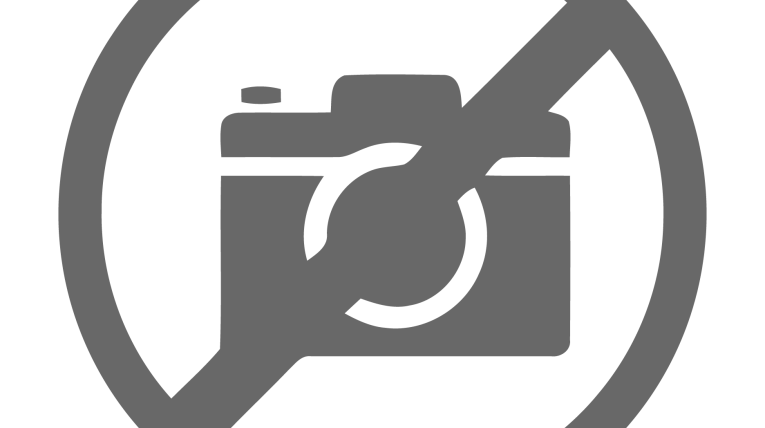
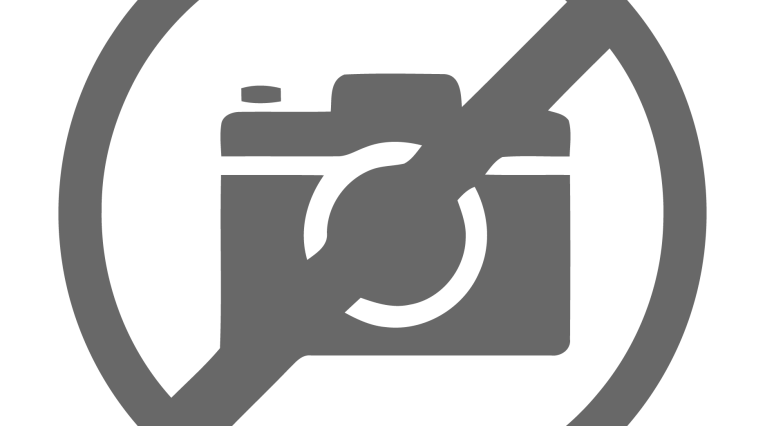
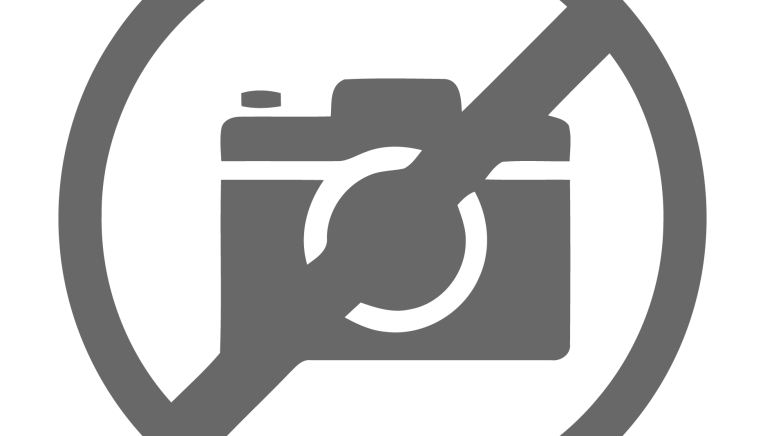

Figure 10 gives the PD.124NR1’s on-axis response indicating a smoothly rising response to about 1.5 kHz followed by some fairly minor break breakup mode peaks at 1.9 kHz and 2.5 kHz just before the low-pass roll-off. Figure 11 displays the on- and off-axis frequency response at 0°, 15°, 30°, and 45°, showing the typical directivity for a 12” woofer. Figure 12 shows the normalized version of Figure 11. Figure 13 shows with the CLIO polar plot (in 10° increments and 1/3 octave smoothing). And finally, Figure 14 displays the two-sample SPL comparisons for the PD.124NR1, showing a close match within ±0.5 dB throughout the operating range up to 1.5 kHz.
For the last remaining series of tests, I employed the Listen, Inc. SoundCheck AudioConnect analyzer and SCM 1/4” microphone to measure distortion and generate time frequency plots (courtesy of my friends at Listen, Inc.). For the distortion measurement, I mounted the PD.124NR1 rigidly in free-air, and set the SPL to 104 dB at 1 m (9.1 V) using a pink noise stimulus, and then measured the distortion with the Listen microphone placed 10 cm from the driver.
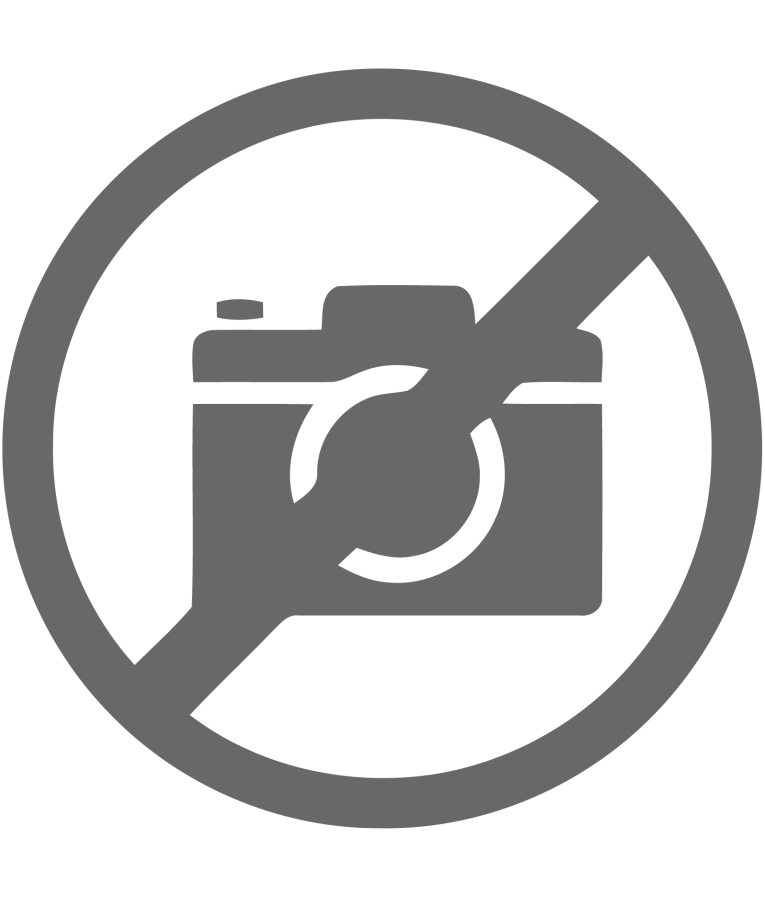
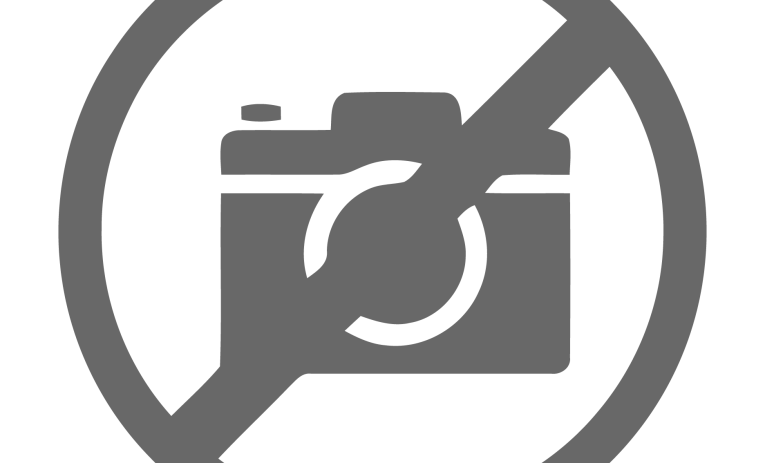
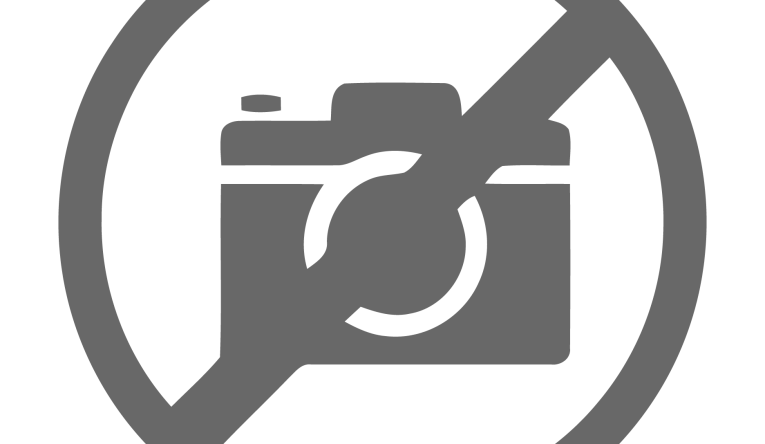
This produced the distortion curves shown in Figure 15. I then used SoundCheck to get a 2.83 V/1 m impulse response for this driver and imported the data into Listen’s SoundMap Time/Frequency software. Figure 16 shows the resulting cumulative spectral decay (CSD) waterfall plot. Figure 17 shows the Wigner-Ville plot (used for its better low-frequency performance).
As I mentioned in the beginning of this explication, it is unusual to have a pro sound driver with a radial-mounted neodymium motor (Aura Systems was the first one, I think). As always I find this woofer, like everything I have seen so far from Precision Devices, to be a very well-crafted driver with a good set of performance trade-offs. For more information, visit www.precision-devices.com. VC
This article was originally published in Voice Coil, September 2019.